Viral Genetics and Immune Evasion: Implications for Vaccine Development
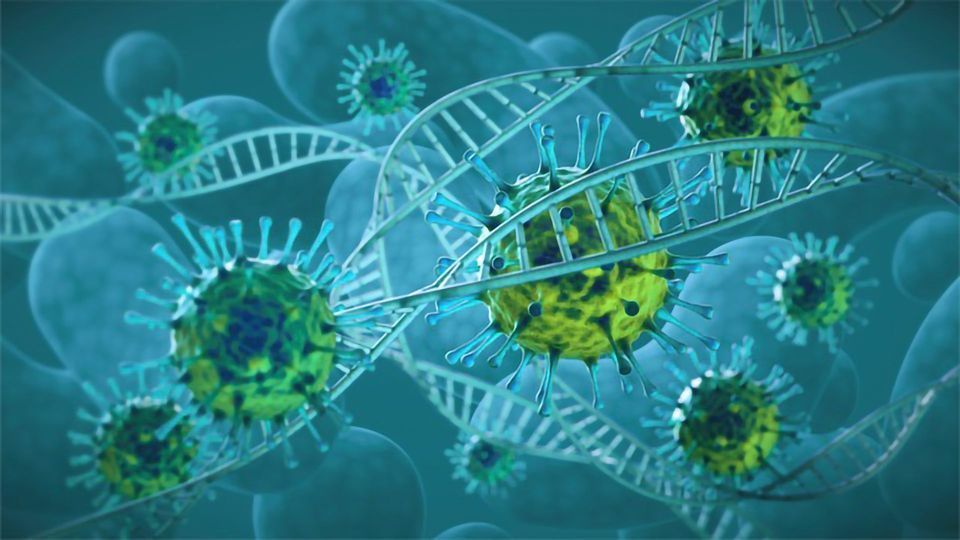
Complete the form below to unlock access to ALL audio articles.
The gold standard of any vaccine is to elicit sterilizing immunity, of which the production of neutralizing antibodies (NAbs) is paramount. Sterilizing immunity prevents a productive viral infection from taking place in the host, in contrast with effective immunity where an infection occurs, but the virus is quickly cleared and severity of disease is much less compared to unvaccinated individuals. While vaccine developers hope to achieve sterilizing immunity, most vaccines also induce T-cell responses. T-cell immunity and immune memory can provide the host with immunological protection against viral infection. However, viral genetic diversity, coupled with immune evasion are an ever-present challenge in the development of a safe and effective vaccine. A virus that exhibits genetic diversity, especially in genes encoding surface-exposed proteins, such as the severe acute respiratory syndrome coronavirus 2 (SARS-CoV-2) spike (S) protein, can evolve and adapt to new host and environmental conditions. Thus, these new viral variants may be able to evade immune protection previously acquired by vaccination or past infection.
While the viral genome is relatively small,1 with every replication there is potential for mistakes to be made. Viruses infect host cells and hijack their machinery to replicate, which can sometimes result in the generation of mutations in the viral genome. The resulting mutations can either be neutral, advantageous or disadvantageous for the virus, depending upon a variety of selective pressures. Selective pressure can come from sources such as the host immune system, the host environment and exposure to vaccines or other antiviral therapeutics. While deleterious mutations will be selected against and removed from the virus populations, mutations that prove beneficial, such as in host range, infectivity or immune evasion may be retained in the viral genome.1
Viruses are genetically diverse biological entities that vary in their genome type (DNA or RNA) and replication methods. RNA viruses have a higher per-site mutation rate, 10-6 to 10-4 new base substitution/nucleotide/cell compared to DNA viruses, 10-8 to 10-6.1 RNA viral genomes are also much more heterogeneous compared to DNA viruses, with double stranded, single stranded and positive or negative sense RNA. Molecular mechanisms involved in introducing genetic diversity into RNA viruses include a lack of proofreading by some RNA-dependent RNA polymerases, recombination and reassortment.1 Although DNA viruses also undergo genetic changes, this article will focus on the changes seen in RNA viruses, such as human immunodeficiency virus-1 (HIV-1), influenza A virus (IAV) and SARS-CoV-2.
Viral genetic diversity
Human immunodeficiency virus-1
A classic example of viral genetic diversity and the challenge it presents to vaccine development is seen in HIV-1. The HIV-1 env gene codes for the envelope glycoprotein which enables viral attachment and entry into host cells. The envelope protein also contains dominant epitopes, excellent targets for NAbs, thus making it a prime candidate as a vaccine antigen. However, the env gene exhibits such high rates of mutation and genetic diversity that a vaccine has yet to be developed that can protect against the various viral subtypes. Because the env gene is so genetically diverse, HIV-1 can be categorized into several groups and subtypes. There are three groups of HIV-1, M, O and N; between these groups ~ 50% env sequence differences are observed.2 Within the M group, there are nine clades with up to 35% env diversity, and each clade has up to 15% variability.2 In addition, the env gene can mutate in a single host, which can then lead to quasispecies that can be unique to a specific host or geographical area.2 It is this incredible diversity that makes the development of HIV-1 vaccines especially difficult. In addition, as this gene continues to come under immune and environmental selective pressures, it will continue to evolve.
Influenza A virus
IAV exhibits diversity focused particularly in genes that code for the surface-exposed proteins hemagglutinin (HA) and neuraminidase (NA). As previously stated, some RNA-dependent RNA polymerases, including that belonging to IAV, do not have a proofreading function. Therefore HA and NA-encoding genes can accumulate point mutations, leading to antigenic drift. Alternatively, due to the segmented nature of the influenza genome, antigenic shift may occur as a result of the wholesale exchange of HA or NA genome segments through reassortment between viruses.3 Antigen shift often occurs in animal reservoirs, such as pigs, when they become concurrently infected with strains from multiple species, for example, a human and an avian IAV. This can then lead to the creation of a new reassortment virus that is able to infect humans but to which we have no preexisting immunity, as seen in previous influenza pandemics.3 Despite IAV’s variable nature, thanks to world surveillance and careful planning, a yearly vaccine is made available based on strains that are circulating or predicted to be circulating. However, having the annual vaccine or contracting a natural infection will not necessarily provide protection against subsequent infection with IAV strains that possess different versions of either HA or NA. Under immune selective pressure, new HA or NA variants that survive the immune response are selected for and become dominant in the IAV population.3
Severe acute respiratory syndrome coronavirus 2
Although the mutation rate of SARS-CoV-2 remains under investigation, it has brought about a unique collaboration among scientist around the world with regard to monitoring genomic changes of the virus, almost in real-time.4 The Global Initiative on Sharing All Influenza Data (GISAID) has over a million SARS-CoV-2 sequences openly available. These sequences, deposited by labs and hospitals worldwide, give a snapshot of viral mutations at various timepoints during this pandemic.4 Harvey, et al. provides a very good review discussing the mutations that have led to the emergence of variants around the world, with divergence away from the original outbreak strain seen as early as April 2020.4 As SARS-CoV-2 continues replicate, almost unchecked in many places around the world, mutations will continue to contribute to the rise of variants.
This viral evolution has been noted by the Centers for Disease Control (CDC) in which variants are defined as “variants of interest”, “variants of concern” and “variants of high consequence”.5 As stated by Dr. Stefan Rothenburg, professor, Department of Medical Microbiology and Immunology at the University of California, Davis, “A lesson learned from the current pandemic is that if a lot of humans are infected with [SARS-CoV-2] it gives the virus a higher chance to adapt to humans or become resistant to vaccines.” Prof. Rothenburg continues, “With the sheer number of people infected, the virus will evolve.”
The S glycoprotein of SARS-CoV-2 mediates host cell attachment, cell entry, is highly immunogenic and a target of NAb, thus is a good antigen for vaccine development.4 However, like the other immunogenic proteins already discussed, there is always a chance that when the gene encoding the S protein is placed under selective pressure, the mutations that develop can lead to changes in immunogenicity, transmissibility, even pathogenicity, all of which impact vaccine development.
Studying the Molecular Mechanism of Viral Replication in Real Time

Viruses interact and hijack host cellular machineries to allow them to replicate and spread. A clear understanding of these host–virus interactions is crucial to uncover the molecular mechanisms underpinning infection and to enable the development of new treatments. Download this app note to discover a fluorescent-based approach that can enable real-time measurement of viral gene expression and be used to study virtually any virus in a 96- or 384- well format.
View App NoteSponsored Content
Immune evasion
Although the HIV-1 envelope glycoprotein can elicit NAbs, they become ineffective as the env gene mutates in response to selective pressure put upon the virus by these NAbs. Thus, the mutations seen in the envelope can lead to loss of an important epitope which results in immune escape.6 These new variants are no longer responsive to NAbs elicited by an earlier “version” of the envelope. HIV-1 immune evasion can also lead to a viral superinfection7 which occurs when an individual is infected by more than one strain of HIV-1.7 Although this may not be common, due to the high genetic diversity of the virus, it is a very real possibility. Superinfection also demonstrates that a natural HIV-1 infection does not protect against the possibility of subsequent infections of an HIV-1 variant.7 These escape mutants pose a challenge to vaccine design in finding a conserved epitope or epitopes that can provide protection against multiple HIV-1 subtypes.7
Two promising vaccine trials are underway to address the challenge of HIV-1 variants; a Phase I study in the United Kingdom by Oxford University and a Phase III study in the United States sponsored by Janssen Vaccines & Prevention B.V. Both trials are using different types of “mosaic” vaccines, incorporating epitopes from two or more HIV-1 variants, to facilitate protection against multiple HIV-1 subtypes.
The genetic diversity of IAV’s HA- and NA-encoding genes provide a type of immune evasion as consequential antigenic changes can render pre-existing antibodies less effective at neutralizing their target or prevent recognition all together. Scientists are also looking at the genetically conserved non-structural protein-1 (NS1), which can help the virus to evade the host immune response by limiting interferon-1 synthesis and limiting pathogen-associated molecular pattern recognition.8 Bypassing or slowing down these innate immune responses allows the virus to replicate unfettered and establish itself in host cells, well ahead of any potential response by the host that could clear the virus from the host cells. To avoid genetic diversity-based immune evasion, researchers are exploring whether evolutionarily conserved proteins of the influenza virus can be considered as vaccine targets, such as matrix-2 protein nucleoprotein and certain conserved HA epitopes.3
The gene encoding the S protein of SARS-CoV-2 has undergone mutations, as evidenced by the new classification by the CDC, as previously discussed above.5 Escape mutants have been selected for due to the immune and environmental pressures acting upon the S gene. These variants can evade the immune response by changing the protein structure so epitopes may be lost or there may be an increase in avidity for the host receptor compared to the NAb.4,9 To tackle SARS-CoV-2, it is imperative to monitor the virus’s genetic changes, especially in regard to those that have the potential to enable the virus to evade the immune response and perhaps bypass the effectiveness of approved vaccines. This is an example of a virus that, while not typically classed as genetically diverse, has evolved before our very eyes in response to high selective pressures.
Molecular Tools To Study Viral Infectious Diseases

In order to fight a virus, it needs to be understood. Advanced studies on the virus life cycle and its interaction with the host are key to identify novel therapeutic targets and develop antiviral drugs. Watch this webinar to learn how the evaluation of binding affinities can support viral research, assist in the identification of novel drugs and identify key functions of viral proteins.
Watch WebinarSponsored Content
New and emerging technologies
Viral genetic diversity, immune evasion and implications for vaccine development cannot be discussed without the mention of new and emerging technologies designed to track these genetic changes in both animal and human viruses. When asked about how important these technologies are for virus research Dr. Adolfo Garcia-Sastre, director of the Global Health and Emerging Pathogens Institute at the Icahn School of Medicine at Mount Sanai, stated, “[They are] very important, it allows the collection of almost real-time genetic information on virus variants becoming more prevalent, allowing rapid testing if the variants evade pre-existing immunity.”
Predicting new emerging infectious diseases is a very difficult task, “In general, it is the holy grail to predict what viruses have the potential to cause outbreaks in humans,” says Prof. Rothenburg. Dr. Garcia-Sastre states that for the scientific community to stay ahead of the next emerging virus is “like predicting the next winning lottery ticket numbers based on the information before.” So, although science may not be able to predict the next emerging viral pathogen – it is a herculean task – changes in the genetics of the many viruses currently infecting animals and humans can be tracked to determine if these changes may lead to changes in immunogenicity, pathogenicity or transmission.
Technologies such as next-generation sequencing (NGS) and deep sequencing allow genetic changes to be monitored at the single nucleotide level. The use of bioinformatics to curate and analyze the sequence data, is paramount in keeping this data organized and available for dissemination. CRISPR technology gives researchers the ability to screen whole viral genomes, looking for genes that may play a role in immune evasion or pathogenesis.10 Lastly, deep mutational scanning gives researchers a tool to interrogate amino acid changes across the genome of both the virus and host, allowing the discovery of interactions between viral and host proteins.11 These are just a few technologies that can be utilized by scientist to take the study of viral genetics, immune evasion and vaccine development forward.
References
1. Sanjuan R, Domingo-Calap P. Genetic diversity and evolution of viral populations. Encyclopedia of Virology. 2021;4(1):53-61. doi: 10.1016/B978-0-12-809633-8.20958-8
2. McBurney SP, Ross TM. Viral sequence diversity: challenges for AIDS vaccine designs. Expert Rev Vaccines. 2008;7(9):1405-1417. doi: 10.1586/14760584.7.9.1405
3. Kim H, Webster RG, Webby RJ. Influenza Virus: Dealing with a drifting and shifting pathogen. Viral Immunol. 2018;31(2):174-183. doi: 10.1089/vim.2017.0141
4. Harvey WT, Carabelli AM, Jackson B, et al. SARS-CoV-2 variants, spike mutations and immune escape. Nat. Rev. Microbiol. 2021;19: 409-424. doi: 10.1038/s41579-021-00573-0
5. SARS-CoV-2 variant classifications and definitions. Centers for Disease Control and Prevention. https://www.cdc.gov/coronavirus/2019-ncov/variants/variant-info.html. Updated Aug 10, 2021. Accessed August 17, 2021.
6. Stefic K, Bouvin-Pley M, Braibant M, Barin F. Impact of HIV-1 diversity on its sensitivity to neutralization. Vaccines. 2019;7(74): 1-14. doi: 10.3390/vaccines7030074
7. Gao, Y, Tian, W, Han, X. and F. Gao. Immunological and virological characteristics of human immunodeficiency virus type 1 superinfection: implications in vaccine design. Front. Med. 2017;11(4): 480–489. doi: 10.1007/s11684-017-0594-8
8. Hale BG, Albrecht RA, Garcia-Sastre A. Innate immune evasion strategies of influenza viruses. Future Microbiol. 2010;5(1):23-41. doi: 10.2217/fmb.09.108
9. Focosi D, Novazzi F, Genoni A, et al. Emergence of SARS-CoV-2 spike protein escape mutation Q493R after treatment for COVID-19. Emerg Infect Diseases. 2021. doi: 10.3201/eid2710.211538
10. Hadidi A. Next-generation sequencing and CRISPR/Cas13 editing in viroid research and molecular diagnostics. Viruses. 2019;11(2):120. doi: 10.3390/v11020120
11. Rothenburg S, Brennan G. Species-specific host-virus interactions: Implications for viral host range and virulence. Trends Microbiol. 2020;28(1):46-56. doi: 10.1016/j.tim.2019.08.007